Sleep
The Memory Function of Sleep
Most of us have often ask “Why do we sleep?” Philosophers have pondered this question for centuries, yet despite decades of research scientists are still striving for an answer. Our understanding of sleep has certainly evolved since the great minds of Plato or Aristotle, who conceived of sleep as resulting from physiological processes such as the digestion of food (Barbera, 2008).
Beginning in the 19th century, empirical studies of the time-course of memory inadvertently pointed toward a special role of sleep in reducing forgetting Opens in new window, but the implications of such findings were later seriously considered, sleep was proposed to play only a passive role in reducing memory interference from external, competing stimuli (Jenkins and Dallenbach, 1924; Van Ormer, 1933).
Fortunately, technological advances such as the electroencephalogram (EEG) led to the blossoming of more objective sleep research (Dement and Vaughan, 2000), allowing scientists to better understand this unique feature of life, including its active role in memory formation and the specific neural processes underlying this function (See How the sleeping brain impacts memory Opens in new window).
Stages of Sleep
The technological advances that led to the first all-night sleep recording in 1953 resulted in the classification of the various stages of sleep still recognized today.
Electrical signals from the brain (electroencephalogram; EEG), eyes (electrooculogram; EOG), and muscles (electromyogram; EMG), collectively making up the polysomnograph (PSG) recording, revealed highly similar patterns of electrical activity in the brain across individuals as they slept. It soon became clear that this pattern included several distinct sleep stages.
- NREM
The first type of sleep became known as NREM or non-rapid-eye-movement sleep and included four stages (1,2,3, and 4) demarking progressively deepening stages of sleep.
- REM
The second type of sleep, REM or rapid-eye-movement sleep, could also be broken down into two different types, known as tonic and phasic events. Tonic REM was characrerized by a lack of eye movements and reduced muscle tone, while phasic REM was characterized by rapid eye movements and minor muscle twitches (Carskadon and Dement, 1989).
Early observations of such recordings allowed researchers to map out the typical night of sleep, which is similar across individuals. At sleep onset, the brain first advances consecutively through stages 1-4.
Stages 3 and 4 together comprise slow-wave sleep (SWS), which is the deepest stage during the sleep cycle and is characterized by high-amplitude, low-frequency EEG patterns. The brain then shifts to REM sleep, which is characterized by low-amplitude, high-frequency EEG patterns, periodic rapid eye movements (REMs), and a drastic reduction in muscle tone.
Figure X Histogram depicting a typical night of sleep. Credit: ResearchGate Opens in new window |
---|
![]() |
Interestingly, the EEG patterns during REM sleep look very similar to patterns obtained during wakefulness, which is why the measurement of eye movements and muscle tone is required to confidently differentiate REM sleep from wakefulness; the EEG alone is often not sufficient.
Following REM sleep, the brain then cycles back through all the stages, repeating this cycle about every 90 minutes throughout the night. However, the distribution of these stages across a night sleep is not entirely even. The first half of the night contains a majority of the night’s SWS, whereas the amount of REM in the second half of the night is nearly doubled from the first (Figure X; Payne, 2011).
It should also be noted that, as of 2007, the American Academy of Sleep Medicine (AASM) has recommended an alteration to these customary sleep stages. According to this change, stage 1 and 2 remain the same, but are referred to as stages N1 and N2, respectively.
However, stages 3 and 4, which were once distinct, are combined into a single stage, noted as N3. Finally, REM sleep is simply referred to as stage R (Iber et al., 2007). Although these changes are recommended and are taught to those currently learning sleep scoring, some researchers still prefer to use the previous method of sleep scoring.
Venterolateral PFC and the Two-Process Model
The left vlPFC refers to the broad region of lateral frontal cortex that is ventral to the inferior frontal sulcus and rostral to promotor cortex (Figure X). Investigation of the function of this region has long provided the strongest evidence in favor of functional specialization within PFC, from the classic studies of language impairment by Paul Broca (e.g., Broca, 1861) to early functional magnetic resonance imaging (fMRI) studies that distinguished subregions within left vlPFC related to the domain of verbal processing (e.g, the semantic versus phonological distinction; Poldrack et al., 1999).
Figure X | Anatomical divisions of vlPFC | Credit: ResearchGate.net Opens in new window |
---|
![]() |
Figure X | Anatomical divisions of vlPFC. (a) Schematic representation of the cytoarchitectonic divisions of the lateral PFC (adapted from Petrides and Pandya, 2002). Labels highlight the anterior vlPFC (parts orbitalis ( ~ Brodmann area [BA] 47)) and mid-vlPFC (pars triangularis (~ BA 45)). (b) Coronal slices from the Montreal Neurological Institute (MNI) canonical brain depict the anatomical boundaries that define mid-vlPFC and anterior vlPFC (reprinted with permission from Badre and Wagner, 2007). Labeled anatomical boundaries are (1) inferior frontal sulcus, (2) insular sulcus, (3) horizontal ramus of the lateral fissure, and (4) orbital gyrus. |
More recent work has focused on functional distinctions within this region as they relate to the cognitive control of memory. Specifically, recent work in our lab and in others has focused on a distinction between controlled retrieval, supported by anterior vlPFC, and post-retrieval selection, supported by mid-vlPFC (Badre and Wagner, 2007).
Here, we will consider the evidence for and against this potential distinction within the left vlPFC. To illustrate the distinction at the process level between controlled retrieval and selection, it is helpful to consider the analogy of searching for specific information on the Internet. For example, consider that you wish to find information about our lab.
First, you need to “hit” our link from the broad, latent associative structure of the web. To do this, you devise a particular keyword to put in your web browser. Of course, some keywords will be more effective than others. For example, searching for “Badre lab” is likely to produce our lab’s website as the top link. However, a less effective search, such as “science lab,” would make it unlikely that you would find the link to our lab without a prohibitive cost in browsing time.
Similar to this example, controlled retrieval refers to strategically guiding the activation of task-relevant information from its latent state. In human memory, controlled retrieval can progress by focusing on or elaborating effective cues and thereby increasing the likelihood that task relevant information is activated from memory.
From this perspective, one means of manipulating controlled retrieval experimentally is to reduce the strength of association between salient cues and target knowledge that would support automatic, cue-driven retrieval. In these cases, a top-down influence can aid in activating relevant knowledge from memory.
Importantly, however, it is very difficult to devise even a pair of keywords in a search engine that produce only a single web link. (In fact, it is rare enough that there is a hobby called “Googlewhacking”, whereby people try to achieve fame by finding pairs of keywords that produce only one hit through GoogleTM.) Thus, once we retrieve information into our browser, we “browse” or further select the links we want from this limited retrieved set.
Though human memory is different in important ways from the Internet, it is a similarly vast, associative structure that uses a form of priority, such as previous co-occurrence, to rank the likelihood that a given representation will be retrieved given a particular cue (Anderson and Milson, 1989; Griffiths, Steyvers, and Firl, 2007).
But, as on the Internet, this associative structure ensures that multiple representations will be retrieved given any cue, and that the highest-ranked representation may not be the one that is needed, given current goals and decision criteria. Thus, it is adaptive if a controlled retrieval system is complemented by an output control system that maintains current decision criteria and selects relevant items from among competitors in working memory Opens in new window.
The process of selecting from among retrieved information is termed post-retrieval selection. From this perspective, manipulations of response or decision criteria or varying the degree of competition among retrieved representations should affect post-retrieval selection.
Multiple lines of evidence support the involvement of the vlPFC in the cognitive control of memory. Functional neuroimaging studies, including using fMRI and positron emission tomography (PET), have repeatedly demonstrated greater activation in vlPFC under conditions of effortful or goal-directed retrieval, such as:
- controlling phonological and semantic representations (e.g., Gold et al., 2005),
- retrieving items with weak versus strong cue support (e.g., Badre et al., 2005),
- overcoming proactive interference (e.g., Ӧztekin and Badre, 2011) and,
- active inhibition of memories (e.g, Anderson et al., 2004).
Moreover, disruption of vlPFC due to neurological damage or disease decreased patients’ ability to select among competing information (Metzler, 2001; Thompson-Scrill et al., 1998). Similarly, intraoperative stimulation (Klein et al., 1997) or application of transcranial magnetic stimulation (Devlin, Mathews, and Rushworth, 2003; Gough, Nobre, and Devlin, 2005) disrupts performance when participants are required to retrieve semantic information.
Thus, in broad terms, vlPFC makes a necessary contribution to cognitive control of memory retrieval. However, an ongoing debate concerns the precise nature of vlPFC contributions to memory retrieval, and its functional organization in support of cognitive control.
Drawing on a wide range of declarative memory paradigms, including tests of both semantic Opens in new window and episodic memory Opens in new window, as well as retrieval during action selection, such as task switching, Badre and Wagner (2007) proposed that distinct subdivisions of the rostral left vlPFC support distinct controlled retrieval and post-retrieval selection processes, associated with the inferior frontal gyrus (IFG) parts orbitalis (~Brodmann area [BA] 47) and parts triangularis (~ BA 45), respectively. These subregions were termed anterior vlPFC and mid-vlPFC, respectively (refer back to Figure X ). We now briefly summarize the evidence that supports this distinction.
The two-process model proposes that anterior vlPFC is activated when memory must be searched in a goal-directed manner (i.e., controlled retrieval; Badre and Wagner, 2007). Accordingly, when bottom-up cues are insufficient to elicit activation of target knowledge (i.e., automatic retrieval), demands on controlled retrieval process increase.
Control can aid retrieval in these contexts by elaborating Opens in new window cues or generating retrieval plans that structure the input to the retrieval system and so make it more likely that relevant information will be retrieved.
Figure Xz | Anatomical representations of mid-vlPFC and anterior vlPFC | Credit: ScienceDirect.com Opens in new window |
---|
![]() |
Figure Xz | Results from manipulations of control during semantic retrieval provide evidence for the two-process model (reprinted with permission from Badre et al., 2005). (a) Contrasts of weak relative to strong associative strength (associative strength) (p < 0.001) and decisions of item similarity based on features (e.g., color) relative to general semantic relatedness (feature specificity) (p < 0.001). (b) Contrasts of associative strength (blue) and feature specificity (red) and their overlap (purple) are rendered on an inflated MNI canonical surface. Anterior vlPFC was sensitive to associative strength, whereas mid-vlPFC was sensitive to both associative strength and feature specificity. |
Consistent with his hypothesis, anterior vlPFC is consistently activated during semantic retrieval tasks in which the association between available cues and target knowledge is weak. For example, deciding that “candle” is semantically related to “flame” is easier and requires less controlled retrieval than deciding that “candle” is related to “halo,” because the association between candle and halo is weak relative to the association between candle and flame. Thus, experiments that manipulate associative strength, based either on pre-experimental norms (Badre et al., 2005; Wagner et al., 2001) or on associations learned during the experimental session (Danker, Gunn, and Anderson, 2008), consistently show greater activation in anterior vlPFC under weak relative to strong associative strength conditions (Figure Xz Opens in new window).
In a way similar to high cue-target association strength, anterior vlPFC shows repetition suppression effects accompanying the increased semantic fluency that follows repetition of an item during a semantic memory task, even when the decision/response level effects are not repeated (Race, Shanker, and Wagner, 2008).
According to the two-process model, mid-vlPFC is activated under conditions in which multiple items are retrieved from memory, but only a subset must be selected for further processing (i.e., post-retrieval selection; Badre and Wagner, 2007). As described above, automatic and controlled retrieval processes can result in the recovery of multiple representations. Thus, post-retrieval selection is needed to resolve competition among the multiple retrieved representations, and to permit selected representation to guide decision and action.
Support for mid-vlPFC and post-retrieval selection comes from several sources. First, mid-vlPFC shows greater activation when participants are asked to decide if two items (e.g., “apple” and “blood”) are similar along a particular dimension, such as color, relative to deciding whether they are generally semantically related to one another regardless of dimension (feature specificity effect) (Badre et al., 2005; Thompson-Schill et al., 1997) (refer back to Figure Xz Opens in new window).
This difference is thought to arise because making the decision along a particular task-relevant dimension requires focusing attention only on the retrieved details relevant to the decision and ignoring any other properties. Notably, anterior vlPFC does not show a difference between specific and general decision conditions (Badre et al., 2005)
Proactive interference (PI) occurs when a prior learned association automatically elicits retrieval of information that competes with a current retrieval task (Anderson and Neely, 1996; Postman and Underwood, 1973). PI during short-term item recognition has consistently been associated with increases activation in mid-vlPFC (Badre and Wagner, 2005; Postle and Brush, 2004; Postle, Brush, and Nick, 2004). PI during short-term item recognition does not consistently produce activation increases in anterior vlPFC. However, as discussed above, other manipulations of PI have been associated with anterior vlPFC activation (Ӧztekin and Badre, 2011).
During lexical decision, an unexpected target produces an interference effect above a neural baseline. This interference effect is thought to be due to competition between information retrieved during preparation for the target and the information that must be retrieved upon encountering the unexpected target. Competition of this type during lexical decision is associated with increased activation in mid-vlPFC.
By contrast, anterior vlPFC shows priming effects consistent with the reduced retrieval demands (Gold et al., 2006). Thus, across these examples, it appears that mid-vlPFC is critical under conditions of competition, presumably when there is a demand to select relevant information for further processing. By contrast, anterior vlPFC is not consistently activated under these circumstances.
Importantly, attempts to directly dissociate anterior and mid-vlPFC are complicated by the fact that, akin to our Internet search analogy, any process of retrieval, be it controlled or automatic, holds the potential for competition. Thus, similar to anterior vlPFC, mid-vlPFC often shows increased activation under conditions requiring controlled retrieval (Badre et al. 2005; Wagner et al., 2001).
And so, though single dissociations are sometimes observed (e.g., Danker, Gunn, and Anderson, 2008), double dissociations are less common. However, if one pits competition against associative strength, it is possible to dissociate these regions. For example, when the number of retrieval cues is small (low overall retrieval) but associative strength is weak, there will be more demands on controlled retrieval and selection.
By contrast, when the number of retrieval cues is large (high overall retrieval) but associative strength is high, this puts greater demands on selection than controlled retrieval. Consistent with this prediction, Badre et al., (2005) directly pitted number of available retrieval cues against associative strength and produced activation in anterior vlPFC but not mid-vlPFC. Thus, crossing the number of retrieval cues (i.e., increasing retrieval demands) with associative strength dissociates anterior from mid-vlPFC; and when this is taken together with the feature specificity described above, this produces a region-by-effect interaction, dissociating anterior and mid-vlPFC (Badre and Wagner, 2007).
In summary, there is evidence both across and within studies for dissociable functions between anterior vlPFC and mid-vlPFC during cognitive control of memory, and these functions can be characterized as controlled retrieval and post-retrieval selection respectively.
Nevertheless, there have been challenges to the two-process model. These have included formal theoretical arguments about whether two processes are required to achieve controlled retrieval and selection function, as opposed to a single-process model that can support both functions (Danker, Gunn, and Anderson, 2008; Thompson-Schill and Botvinick, 2006). These models make clear that a single process could achieve these two functions. However, it would seem difficult for a single-process model to account for the empirical dissociation between these processes.
There has also been some debate about the nature of the relationship between anterior vlPFC and controlled retrieval, and whether the manipulation of associative strength actually reflects the domain of information being retrieved, such as retrieval of abstract semantics (Goldberg et al., 2007). Again, however, a strictly domain-based account appears too difficult to reconcile with the broader data supporting the controlled retrieval hypothesis, such as the observation of activation in anterior vlPFC when retrieving weak, arbitrary paired associations (Danker, Gunn, and Anderson, 2008).
See also:
- The Human HippocampusOpens in new window
- Role of The Hippocampus In RetrievalOpens in new window
- Role of the Parietal Cortex in RetrievalOpens in new window
- Role of the Prefrontal Cortex in RetrievalOpens in new window
- Role of the Amygdala in EmotionOpens in new window
- Anterior Temporal Regions: Amodal Storage of Semantic InformationOpens in new window
- Medial Temporal Lobe (MTL) Contributions to MemoryOpens in new window
Cited references in the book include:
- Allan, K., Wilding, E.L., and Rugg, M.D. (1998). Electrophysiological evidence for dissociable processes contributing to recollection. Acta Psychologica, 98 (2-3), 231-52. doi: 10.1016/S0001-6918(97)00044-9.
- Anderson, J. R., and Milson, R. (1989). Human memory: an adaptive perspective. Psychological Review, 96 (4), 703-719. doi: 10.1037/0033-2595X.96.4.703.
- Anderson, M.C., and Neely, J.H. (1996). Interference and inhibition in memory retrieval. In Memory Handbook of Perception and Cognition (ed. E. L. Bjork and R. A. Bjork). San Diego, CA: Academic Press, pp. 237-313.
- Anderson, M.C., Ochsner, K.N., Kuhl., et al., (2004). Neural systems underlying the suppression of unwanted memories. Science, 303 (5655), 232-235. doi: 10.1126/science.1089504.
- Badre, D., Poldrack, R.A. Pare-Blagoev, E.J., et al., (2005). Dissociable controlled retrieval and generalized selection mechanisms in ventrolateral prefrontal cortex. Neuron, 47 (6), 907-918. doi: 10.1016/j.neuron.2005.07.023.
- Badre, D., and Wagner, A.D. (2005). Frontal lobe mechanisms that resolve proactive interference. Cerebral Cortex, 15 (12), 2003-2012. doi:10.1093/cercor/bhi075.
- Badre, D., and Wagner, A.D. (2007). Left ventrolateral prefrontal cortex and the cognitive control of memory. Neuropsychologia 45 (13), 28883-2901. doi: 10.1016./j.neuropsychologia.2007.06.015.
- Barredo, J., Ӧztekin, I., and Badre, D. (2013). Ventral fronto-temporal pathway supporting cognitive control of eipisodic memory retrieval. Cerebral Cortex, published online October 31, 2013. doi: 10.1093/cercor/bht291.
- Benjamin, A.S. (2007). Memory is more than just remembering: strategic control of encoding, accessing memory, and making decisions. In The Psychology of Learning and Motivation, Volume 48: Skill and Strategy in Memory Use (ed. A. S. Benjamin and B. H. Ross). New York, NY: Elsevier, pp. 175-223.
- Blumenfeld, R.S., and Ranganath, C. (2007). Prefrontal cortex and long-term memory encoding: an integrative review of findings from neuropsychology and neuroimaging. Neuroscientist, 13 (3), 280-291. doi: 10.1177/1073858407299290.
- Ranganath, C. (2010). A unified framework for the functional organization of the medial temporal lobes and the phenomenology of episodic memory. Hippocapus, 20 (11), 1263-1290. doi: 10.1002/hipo.20852.
- Ranganath, C. and Rainer, C. (2003). Neural mechanisms for detecting and remembering novel events. Nature Review Neuroscience, 4 (3), 193-202. doi: 10.1038/nrn1052.
- Schacter, D.L, and Slotnick, S.D. (2004). The cognitive neuroscience of memory distortion. Neuron, 44 (1), 149-160. doi: 10.1016/j.neuron.2004.08.017.
- Scoville, W.B., and Milner, B. (1957). Loss of recent memory after bilateral hippocampal lesions. Journal of Neurology, Neurosurgery, and Psychiatry, 20 (1), 11-21. doi: 10.1136/jnnp.20.1.11.
- Tulving, E. (1985). Memory and consciousness. Canadian Psychology/Psychologie Canadienne, 26 (1), 1-12. doi: 10.1037/h0080017.
- Vargha-Khadem, F., Gadian, D.G., Watkins, K.E., et al. (1997). Differential effects of early hippocampal pathology on episodic and semantic memory. Science, 277 (5324), 376-380. doi:10.1126/science.277.5324.376.
- Yonelinas, A.P. (2002). The nature of recollection and familiarity: a review of 30 years of research. Journal of Memory and Language, 46 (3), 441-517. doi: 10.1006/jmla.2002.2864.
- Yonelinas, A.P., and Jacoby, L.L. (2012). The process-dissociation approach two decades later: convergence, boundary conditions, and new directions. Memory and Cognition, 40 (5), 663-680. doi:10.3758/s13421-012-0205-5.
- Yonelinas, A.P., and Parks, C.M. (2007). Receiver operating characteristics (ROCs) in recognition memory: areview . Psychological Bulletin, 133 (5),800-832. doi: 10.1037/0033-2909.133.5.800.
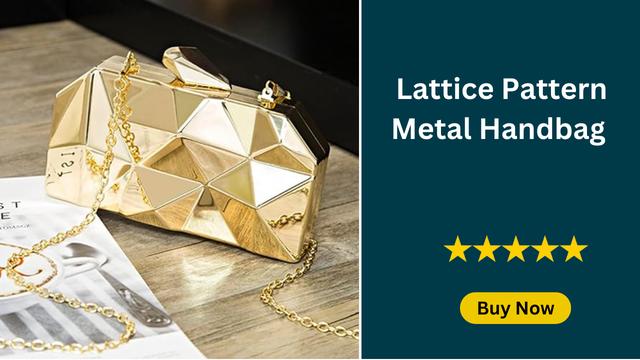